The geological overview of Yellowstone Caldera differs from that of Valles Caldera. Yellowstone Caldera is a product of a hotspot that is located beneath the North American Plate; the hotspot has been active for the last 16.5 million years (Lowenstern et al., 2006). As the North American Plate moved in a southwestern direction at a steady rate over the stationary hotspot, it created a linear string of volcanic features from northern Nevada through the Snake River Plain to its present-day location in the northwestern part of Wyoming (Smith and Siegel, 2000). The Yellowstone volcanic field contains three calderas formed by magmatic activity associated with a mantle hotspot (Lowenstern et al., 2006). The youngest caldera, Yellowstone Caldera, formed 640,000 YBP, and currently has an active hydrothermal system and resurgent domes in the central parts of the caldera (Fig. 2).
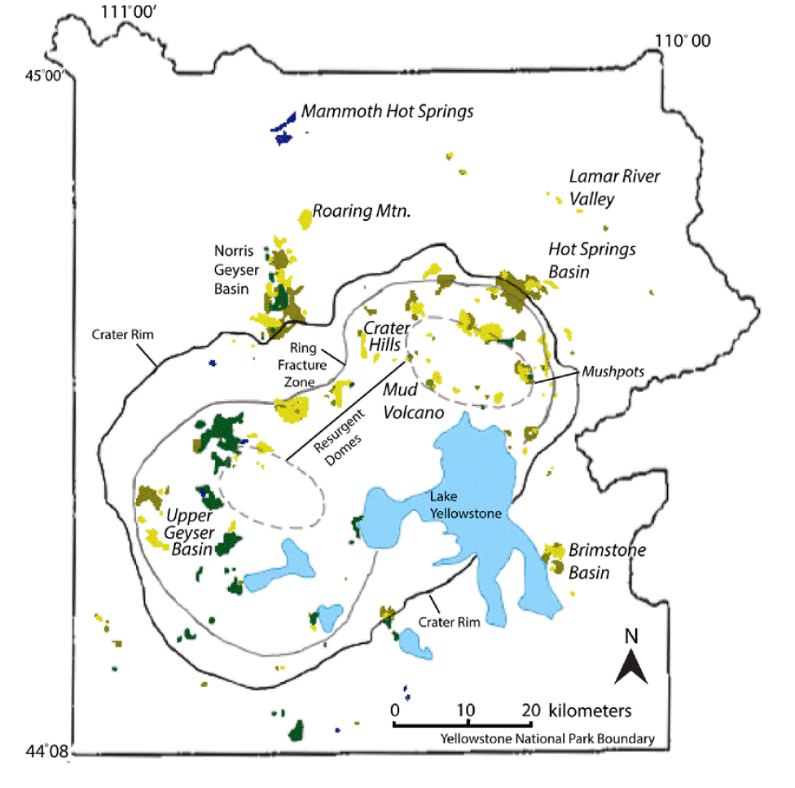
Figure 2: Location of soil chemistries and high activity areas within Yellowstone caldera (Figure modified from Werner and Brantley, 2003). Light and dark yellow indicates acid-sulfate soil, green indicates neutral-chloride soil, and dark blue indicates travertine soil. Thermal areas previously studied by others are italicized.
The magma body is relatively shallow, which results in high surface heat flow and abundant earthquakes. Geothermal features such as fumaroles and geysers occur in an area of ~ 70 km2. Published data indicate that Yellowstone Caldera releases over 45,000 tons of CO2 per day (Werner and Brantley, 2003).
The Yellowstone Plateau Volcanic Field is an open geothermal system where high temperature, CO2-rich magmatic gases mix with low temperature, 4He-rich crustal gases and gases derived from shallow boiling of meteoric-water-derived geothermal liquid (Lowenstern et al., 2015). The gases are often not in equilibrium with liquids due to rapid open-system processes, especially in acid-sulfate terrains, which may cause temperature estimates from geothermometry to be inaccurate. Different thermal areas have distinct gas ratios and isotopic compositions reflecting different sources. For example, Mud Volcano has a strong mantle helium component, while areas in the east side of the park such as Hot Spring Basin have a significant crustal helium contribution (Hurwitz and Lowenstern, 2014).
Similar to Valles Caldera, acid-sulfate springs and mudpots occur at higher elevations in Yellowstone Caldera, while compositionally diverse neutral-Cl, SiO2-rich boiling and near-boiling springs are found at lower elevations (Chiodini et al., 2012). Fournier (Fournier, 1989) argued that the neutral-Cl waters all derived from a parent with 400 mg/L Cl and temperature of 340°C mixed with cold, dilute meteoric water. However, this model does not explain the high He concentrations and high variation in He isotope ratios. More recent work concludes that high temperature gases in Yellowstone Caldera result from mixing between a deep, mostly meteoric-derived water with magma-derived solutes and gases at a temperature of ~340°C (mantle component) and a shallow, 4He-rich, dominantly meteoric water at ~170°C (crustal component) (Chiodini et al., 2012). The estimated composition of the magmatic fluid has 4He/40Ar and 3He/4He values consistent with a mantle plume source.
Werner and Brantley (Werner and Brantley, 2003) interpolated their spot measurements of diffuse surface flux using soil chemistry and temperature. CO2 fluxes are higher in hydrothermally altered soils. The soil classification is the same as the classification for geothermal fluids: neutral-chloride, travertine-precipitating, and acid sulfate regions where fluxes are high due to their association with fracture zones (>96% of CO2 derives from the acid-sulfate regions). As for Valles Caldera, carbon isotopes alone are not useful for identifying the source of CO2 due to compositional overlap of potential sources (in this case mantle hotspot and Madison limestone). Combining δ13C-CO2 with He isotopes using the method of Sano and Marty (Sano and Marty, 1995) led to estimates of ~40% of CO2 from sedimentary sources and ~60% from magmatic sources (Werner and Brantley, 2003). The total diffuse CO2 flux is 3.7 x 1011 mol/y, between 8 and 16% of global plume CO2 emissions. At Yellowstone Caldera the greatest need is for time-series measurements of gas fluxes to provide constraints on timescales of gas migration through the crust, especially during and after seismic events (Lowenstern et al., 2015).
References
Chiodini G., Caliro S., Lowenstern J. B., Evans W. C., Bergfeld D., Tassi F. and Tedesco D. (2012) Insights from fumarole gas geochemistry on the origin of hydrothermal fluids on the Yellowstone Plateau. Geochim. Cosmochim. Acta 89, 265–278. Available at: http://www.sciencedirect.com/science/article/pii/S0016703712002566.
Fournier R. O. (1989) Geochemistry and dynamics of the Yellowstone National Park hydrothermal system. Annu. Rev. Earth Planet. Sci. 17, 13–53.
Hurwitz S. and Lowenstern J. B. (2014) Dynamics of the Yellowstone hydrothermal system. Rev. Geophys. 52, 375–411. Available at: http://dx.doi.org/10.1002/2014RG000452.
Lowenstern J. B., Bergfeld D., Evans W. C. and Hunt A. G. (2015) Origins of geothermal gases at Yellowstone. J. Volcanol. Geotherm. Res. 302, 87–101. Available at: http://dx.doi.org/10.1016/j.jvolgeores.2015.06.010.
Lowenstern J. B., Smith R. B. and Hill D. P. (2006) Monitoring super-volcanoes: geophysical and geochemical signals at Yellowstone and other large caldera systems. Philos. Trans. R. Soc. A Math. Phys. Eng. Sci. 364, 2055 LP-2072. Available at: http://rsta.royalsocietypublishing.org/content/364/1845/2055.abstract.
Sano Y. and Marty B. (1995) Origin of carbon in fumarolic gas from island arcs. Chem. Geol. 119, 265–274.
Smith R. B. and Siegel L. J. (2000) Windows into the Earth: The Geologic Story of Yellowstone and Grand Teton National Parks., Oxford University Press, New York.
Werner C. and Brantley S. (2003) CO2 emissions from the Yellowstone volcanic system. Geochemistry, Geophys. Geosystems 4, n/a-n/a. Available at: http://dx.doi.org/10.1029/2002GC000473.